Immiscible C-H-O fluids formed at subduction zone conditions
Affiliations | Corresponding Author | Cite as | Funding informationKeywords: mantle fluids, fluid immiscibility, carbon cycle, subduction zone, experiments
-
Share this article
Article views:10,211Cumulative count of HTML views and PDF downloads.
- Download Citation
-
Rights & Permissions
Abstract

Figures and Tables
![]() Table 1 Summary of experimental conditions and results. |
![]() Figure 1 Representative synthetic fluid inclusions in quartz formed at 0.2 to 2.5 GPa, 600 to 700 °C, and fO2 buffered by the Fe-FeO to the Re-ReO2 buffer. (a) At 0.2 GPa, only one type of fluid inclusion was observed and these fluid inclusions show a constant vapour/liquid ratio. At 1.5 and 2.5 GPa, three different types of fluid inclusions were observed: (b, c, d) Type-1 fluid inclusions are water-rich and show weak optical contrast to quartz; (b, c, d, e) Type-2 fluid inclusions are nearly pure gases and show strong optical contrast to quartz; and (c) Type-3 fluid inclusions are mixtures of Type-1 and Type-2 fluids and show variable vapour/liquid ratios. (d, f) Graphite occurs in fluid inclusions and also as solid inclusions in quartz. The coexistence of Type-1 and Type-2, together with Type-3, fluid inclusions at 1.5 and 2.5 GPa demonstrates that immiscible fluid phases must have occurred during the run. The red spots refer to the laser spots of Raman measurements, and representative Raman spectra are shown in Figure 2. |
![]() Figure 2 Representative Raman spectra of synthetic fluid inclusions in quartz formed at 0.2 to 2.5 GPa, 600 to 700 °C, and fO2 buffered by the Fe-FeO to the Re-ReO2 buffer. At 0.2 GPa, 700 °C, and fO2 at the FMQ buffer, CO2 was the only detectable carbon species in fluids. At 2.5 GPa, (a) Type-1 fluid inclusions are rich in H2O but with detectable CH4+C2H6, CH4+CO2, and CO2; (b) the corresponding Type-2 fluid inclusions are nearly pure CH4+H2+C2H6, CH4+CO2, and CO2. Note that the gas species vary as a function of fO2. Also note that the fO2 in run LY19 (ReReO2-700 °C-2.5 GPa) buffered by Re-ReO2 may be close to the C-CO2 buffer (see Supplementary Information). The Raman spectra of one graphite inclusion in quartz is also shown in panel (a). The peak positions are about 1285 cm-1 and 1388 cm-1 for CO2, 2917 cm-1 for CH4, 4150 cm-1 for H2, 2956 cm-1 for C2H6, and 3072 cm-1 for aromatics. The small peaks at about 2331 cm-1 and 1555 cm-1 could be N2 and O2 from the air, respectively. Aromatics might be produced during quench. |
Table 1 | Figure 1 | Figure 2 |
Supplementary Figures and Tables
![]() Figure S-1 Sketch of the four experimental capsule setups used in this study. (a, b) Panels are for the experiments performed at 0.2 GPa. (c, d) Panels are for the experiments performed at 1.5 to 2.5 GPa. The wall thickness of Au or Pt capsule in panels (a, b) and the wall thickness of the inner Pt95Rh05 capsule in panels (c, d) are about 0.15 mm and 0.2 mm, respectively. FMQ = fayalite + magnetite + quartz. See text for more details. |
Figure S-1 |
top
Introduction
Thermodynamic simulations and experimental studies demonstrate that carbonate minerals are stable along most subduction P-T paths and up to 90 % of the carbon in subduction zones should be subducted into the deep mantle (Kerrick and Connolly, 2001
Kerrick, D., Connolly, J. (2001) Metamorphic devolatilization of subducted marine sediments and the transport of volatiles into the Earth's mantle. Nature 411, 293-296.
; Dasgupta and Hirschmann, 2010Dasgupta, R., Hirschmann, M.M. (2010) The deep carbon cycle and melting in Earth's interior. Earth and Planetary Science Letters 298, 1-13.
). However, there is compelling evidence suggesting that about 20−80 % of carbon is removed from the downgoing slab and returned to Earth’s surface by arc volcanism (Dasgupta and Hirschmann, 2010Dasgupta, R., Hirschmann, M.M. (2010) The deep carbon cycle and melting in Earth's interior. Earth and Planetary Science Letters 298, 1-13.
; Johnston et al., 2011Johnston, F.K., Turchyn, A.V., Edmonds, M. (2011) Decarbonation efficiency in subduction zones: Implications for warm Cretaceous climates. Earth and Planetary Science Letters 303, 143-152.
; Kelemen and Manning, 2015Kelemen, P.B., Manning, C.E. (2015) Reevaluating carbon fluxes in subduction zones, what goes down, mostly comes up. Proceedings of the National Academy of Science USA 112, E3997-E4006.
). Several models have been proposed to account for the mechanisms of transferring slab carbon to the mantle wedge. Among these are metamorphic decarbonation (Poli et al., 2009Poli, S., Franzolin, E., Fumagalli, P., Crottini, A. (2009) The transport of carbon and hydrogen in subducted oceanic crust: An experimental study to 5 GPa. Earth and Planetary Science Letters 278, 350-360.
), carbonate dissolution in the dehydrating fluids as gas molecules and/or ionic carbon species (Caciagli and Manning, 2003Caciagli, N.C., Manning, C.E. (2003) The solubility of calcite in water at 6–16 kbar and 500–800 °C. Contributions to Mineralogy and Petrology 146, 275-285.
; Frezzotti et al., 2011Frezzotti, M., Selverstone, J., Sharp, Z., Compagnoni, R. (2011) Carbonate dissolution during subduction revealed by diamond-bearing rocks from the Alps. Nature Geoscience 4, 703-706.
; Sverjensky et al., 2014Sverjensky, D.A., Stagno, V., Huang, F. (2014) Important role for organic carbon in subduction-zone fluids in the deep carbon cycle. Nature Geoscience 7, 909-913.
), infiltrating fluids induced decarbonation (Kerrick and Connolly, 2001Kerrick, D., Connolly, J. (2001) Metamorphic devolatilization of subducted marine sediments and the transport of volatiles into the Earth's mantle. Nature 411, 293-296.
; Gorman et al., 2006Gorman, P.J., Kerrick, D.M., Connolly, J.A.D. (2006) Modeling open system metamorphic decarbonation of subducting slabs. Geochemistry, Geophysics, Geosystems 7, doi: 10.1029/2005gc001125.
; Bebout and Penniston-Dorland, 2016Bebout, G.E., Penniston-Dorland, S.C. (2016) Fluid and mass transfer at subduction interfaces—The field metamorphic record. Lithos 240, 228-258.
), and decarbonation accompanied by silicate precipitation via fluid-carbonate reaction (Ague and Nicolescu, 2014Ague, J., Nicolescu, S. (2014) Carbon dioxide released from subduction zones by fluid-mediated reactions. Nature Geoscience 7, 355-360.
). Nevertheless, the respective contribution of these models in transferring slab carbon to the mantle wedge is unclear. For example, Galvez et al. (2013)Galvez, M.E., Beyssac, O., Martinez, I., Benzerara, K., Chaduteau, C., Malvoisin, B., Malavieille, J. (2013) Graphite formation by carbonate reduction during subduction. Nature Geoscience 6, 473-477.
proposed that the reaction between serpentinite-derived fluids and carbonates may cause the formation of graphite and provide an important mechanism for the deep subduction of carbon; whereas Ague and Nicolescu (2014)Ague, J., Nicolescu, S. (2014) Carbon dioxide released from subduction zones by fluid-mediated reactions. Nature Geoscience 7, 355-360.
showed that fluid-carbonate reaction may cause significant decarbonation and efficient transfer of slab carbon to the mantle wedge.Subduction zones are undoubtedly the locations where the Earth’s mantle is most enriched in C-H-O fluids. However, despite significant progress in experiments and thermodynamic modelling on C-H-O fluids (e.g., Churakov and Gottschalk, 2003
Churakov, S., Gottschalk, M. (2003) Perturbation theory based equation of state for polar molecular fluids: II. Fluid mixtures. Geochimica et Cosmochimica Acta 67, 2415-2425.
; Heinrich, 2007Heinrich, W. (2007) Fluid immiscibility in metamorphic rocks. Reviews in Mineralogy and Geochemistry 65, 389-430.
; Zhang and Duan, 2009Zhang, C., Duan, Z. (2009) A model for C–O–H fluid in the Earth’s mantle. Geochimica et Cosmochimica Acta 73, 2089-2102.
; Manning et al., 2013Manning, C.E., Shock, E.L., Sverjensky, D.A. (2013) The Chemistry of Carbon in Aqueous Fluids at Crustal and Upper-Mantle Conditions: Experimental and Theoretical Constraints. Reviews in Mineralogy and Geochemistry 75, 109-148.
), our understanding of the nature of mantle C-H-O fluids is heavily hampered by the lack of experiments at relevant conditions. Previous studies assumed that H2O and gases of CH4, CO2, or H2 are fully miscible at conditions of most of the crust and the entire mantle (e.g., Heinrich, 2007Heinrich, W. (2007) Fluid immiscibility in metamorphic rocks. Reviews in Mineralogy and Geochemistry 65, 389-430.
; Manning et al., 2013Manning, C.E., Shock, E.L., Sverjensky, D.A. (2013) The Chemistry of Carbon in Aqueous Fluids at Crustal and Upper-Mantle Conditions: Experimental and Theoretical Constraints. Reviews in Mineralogy and Geochemistry 75, 109-148.
). The addition of salt, such as NaCl or CaCl2, may yield an extensive region of immiscibility in the H2O-CH4/CO2-salt system at crustal conditions (Bowers and Helgeson, 1983Bowers, T.S., Helgeson, H.C. (1983) Calculation of the thermodynamic and geochemical consequences of nonideal mixing in the system H2O-CO2-NaCl on phase-relations in geologic systems - Metamorphic equilibria at high-pressures and temperatures. American Mineralogist 68, 1059-1075.
; Heinrich, 2007Heinrich, W. (2007) Fluid immiscibility in metamorphic rocks. Reviews in Mineralogy and Geochemistry 65, 389-430.
; Manning et al., 2013Manning, C.E., Shock, E.L., Sverjensky, D.A. (2013) The Chemistry of Carbon in Aqueous Fluids at Crustal and Upper-Mantle Conditions: Experimental and Theoretical Constraints. Reviews in Mineralogy and Geochemistry 75, 109-148.
). However, at deep crustal conditions the salt concentration may need to be more than 20 wt. % to yield fluid immiscibility if CO2 or CH4 mole fraction in the system is less than 0.5 (Johnson, 1991Johnson, E.L. (1991) Experimentally determined limits for H2O-CO2-NaCl immiscibility in granulites. Geology 19, 925-928.
; Shmulovich et al., 2004Shmulovich, K.I., Graham, C.M. (2004) An experimental study of phase equilibria in the systems H2O-CO2-CaCl2 and H2O-CO2-NaCl at high pressures and temperatures (500-800 °C, 0.5-0.9 GPa): geological and geophysical applications. Contributions to Mineralogy and Petrology 146, 450-462.
). Two previous studies showed that pressure can also expand the miscibility gap of the H2O-H2 and H2O-CH4 systems (Churakov and Gottschalk, 2003Churakov, S., Gottschalk, M. (2003) Perturbation theory based equation of state for polar molecular fluids: II. Fluid mixtures. Geochimica et Cosmochimica Acta 67, 2415-2425.
; Bali et al., 2013Bali, E., Audétat, A., Keppler, H. (2013) Water and hydrogen are immiscible in Earth's mantle. Nature 495, 220-222.
), but presently no experiments have been performed on the C-H-O-salt system at mantle conditions. Here I show experimentally that in the presence of 3 wt. % NaCl, H2O and gases of CH4+H2, CH4+CO2, or CO2 can coexist as two separate, immiscible fluids at subduction zone conditions, and I suggest that this immiscibility may play an important role in the deep carbon cycle.top
Results
High-pressure experiments were designed to entrap C-H-O fluids as fluid inclusions in quartz crystals at 0.2 to 2.5 GPa, 600 to 700 °C, and oxygen fugacity (fO2 hereafter) buffered by the Fe-FeO, Co-CoO, FMQ, Ni-NiO, or Re-ReO2 buffer (see Supplementary Information). The starting C-H-O solution was prepared by mixing 78.5 wt. % distilled water, 3 wt. % NaCl, and 18.5 wt. % formic acid (HCO2H), but the C-H-O fluid speciation present during the run primarily depends on the fO2 imposed. The results show that at 0.2 GPa, 700 °C, and fO2 buffered by FMQ and Ni-NiO (Table 1), only one type of fluid inclusion exists in the quartz crystal (Fig. 1a), and CO2 is the only detectable carbon species (Fig. 2a). At 1.5 to 2.5 GPa and 600 to 700 °C (Table 1), three types of fluid inclusions were found in each run. Type-1 are light-coloured fluid inclusions with weak optical contrast to the surrounding quartz (Fig. 1b,c,d). In Type-1 fluid inclusions, if a vapour bubble occurs in the fluid inclusions, the vapour/liquid ratio is nearly constant (Fig. 1b). Type-2 are dark-coloured fluid inclusions with strong optical contrast to the surrounding quartz (Fig. 1b,c,d,e). Raman measurements show that Type-1 fluid inclusions are H2O-rich with small but detectable amounts of gases of CH4+C2H6, CH4+CO2, or CO2 (Fig. 2a). The gas species vary from CH4+C2H6 to CO2 at fO2 varying from the Fe-FeO to the Re-ReO2 buffer. The corresponding Type-2 fluid inclusions are pure gases of H2+CH4+C2H6, CH4+CO2, or CO2 without detectable water at room temperatures (Fig. 2b), although a thin film of water may exist on the inner surface of the fluid inclusions (Berkesi et al., 2009
Berkesi, M., Hidas, K., Guzmics, T., Dubessy, J., Bodnar, R., Szabo, C., Vajna, B., Tsunogae, T. (2009) Detection of small amounts of H2O in CO2-rich fluid inclusions using Raman spectroscopy. Journal of Raman Spectroscopy 40, 1461-1463.
; Frezzotti et al., 2012Frezzotti, M.L., Ferrando, S., Tecce, F., Castelli, D. (2012) Water content and nature of solutes in shallow-mantle fluids from fluid inclusions. Earth and Planetary Science Letters 351-352, 70-83.
). The coexistence of Type-1 and Type-2 fluid inclusions demonstrates that two separate, immiscible fluid phases must have occurred during the run. The third type of fluid inclusion contains a vapour bubble but shows different vapour/liquid ratios (Fig. 1c). Type-3 fluid inclusions are interpreted to be the entrapment of mixed Type-1 and Type-2 fluids. In addition, graphite always occurs in fluid inclusions and also as solid inclusions in quartz crystals from the runs buffered by Co-CoO, Ni-NiO, and Re-ReO2 (Figs. 1b,d,f; 2a). The occurrence of graphite inclusions in quartz crystals indicates that this graphite must be produced during the run probably by the reactionEq. 1
CH4 + CO2 = 2C (graphite) + 2H2O
Eq. 2
CO2 + 2H2 = C (graphite) + 2H20
Table 1 Summary of experimental conditions and results.
Run No | P | T | Duration | Oxygen buffer | Immiscible fluids | Graphite saturation | aFluid composition | |
| GPa | °C | days | | ||||
LY13 | 0.2 | 700 | 3 | FMQ | No | No | H2O-CO2 | |
LY14 | 0.2 | 700 | 3 | Ni-NiO | No | No | H2O-CO2 | |
| Type-1 | Type-2 | ||||||
LY01 | 1.5 | 600 | 3 | Fe-FeO | Yes | No | H2O-CH4-C2H6 | CH4-H2-C2H6 |
LY02 | 2.5 | 600 | 3 | Fe-FeO | Yes | No | H2O-CH4-C2H6 | CH4-H2-C2H6 |
LY05 | 2.5 | 600 | 2 | Ni-NiO | Yes | Yes | H2O-CH4-CO2 | CH4-CO2 |
LY08 | 2.5 | 600 | 6 | Ni-NiO | Yes | Yes | H2O-CH4-CO2 | CH4-CO2 |
LY04 | 2.5 | 700 | 2 | Ni-NiO | Yes | Yes | H2O-CH4-CO2 | CH4-CO2 |
LY21 | 2.5 | 700 | 2.5 | Co-CoO | Yes | Yes | H2O-CH4-CO2 | CH4-CO2 |
LY19 | 2.5 | 700 | 2 | Re-ReO2 | Yes | Yes | H2O-CO2 | CO2 |
a The fluid composition was determined by Raman spectroscopy. In each run, 3 wt. % NaCl was added to the starting solution.
Note that the oxygen fugacity in run LY19 may be close to the C-CO2 buffer. See text for more details.
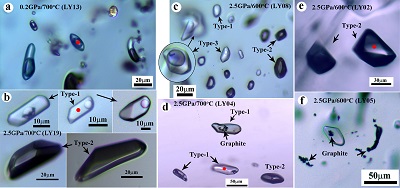
Figure 1 Representative synthetic fluid inclusions in quartz formed at 0.2 to 2.5 GPa, 600 to 700 °C, and fO2 buffered by the Fe-FeO to the Re-ReO2 buffer. (a) At 0.2 GPa, only one type of fluid inclusion was observed and these fluid inclusions show a constant vapour/liquid ratio. At 1.5 and 2.5 GPa, three different types of fluid inclusions were observed: (b, c, d) Type-1 fluid inclusions are water-rich and show weak optical contrast to quartz; (b, c, d, e) Type-2 fluid inclusions are nearly pure gases and show strong optical contrast to quartz; and (c) Type-3 fluid inclusions are mixtures of Type-1 and Type-2 fluids and show variable vapour/liquid ratios. (d, f) Graphite occurs in fluid inclusions and also as solid inclusions in quartz. The coexistence of Type-1 and Type-2, together with Type-3, fluid inclusions at 1.5 and 2.5 GPa demonstrates that immiscible fluid phases must have occurred during the run. The red spots refer to the laser spots of Raman measurements, and representative Raman spectra are shown in Figure 2.

Figure 2 Representative Raman spectra of synthetic fluid inclusions in quartz formed at 0.2 to 2.5 GPa, 600 to 700 °C, and fO2 buffered by the Fe-FeO to the Re-ReO2 buffer. At 0.2 GPa, 700 °C, and fO2 at the FMQ buffer, CO2 was the only detectable carbon species in fluids. At 2.5 GPa, (a) Type-1 fluid inclusions are rich in H2O but with detectable CH4+C2H6, CH4+CO2, and CO2; (b) the corresponding Type-2 fluid inclusions are nearly pure CH4+H2+C2H6, CH4+CO2, and CO2. Note that the gas species vary as a function of fO2. Also note that the fO2 in run LY19 (ReReO2-700 °C-2.5 GPa) buffered by Re-ReO2 may be close to the C-CO2 buffer (see Supplementary Information). The Raman spectra of one graphite inclusion in quartz is also shown in panel (a). The peak positions are about 1285 cm-1 and 1388 cm-1 for CO2, 2917 cm-1 for CH4, 4150 cm-1 for H2, 2956 cm-1 for C2H6, and 3072 cm-1 for aromatics. The small peaks at about 2331 cm-1 and 1555 cm-1 could be N2 and O2 from the air, respectively. Aromatics might be produced during quench.
top
Discussion
The presence of C-H-O fluid immiscibility at 1.5 and 2.5 GPa, and the absence of C-H-O fluid immiscibility at 0.2 GPa demonstrates that pressure significantly expands the C-H-O fluid miscibility gap. This is in good agreement with one recent experimental study (Bali et al., 2013
Bali, E., Audétat, A., Keppler, H. (2013) Water and hydrogen are immiscible in Earth's mantle. Nature 495, 220-222.
), which shows that the critical temperature of the H2O-H2 system increases from below 400 °C at 0.25 GPa to 1050 °C at 2.6 GPa. Theoretical calculations also indicate the expansion of the fluid miscibility gap with pressure; Churakov and Gottschalk (2003)Churakov, S., Gottschalk, M. (2003) Perturbation theory based equation of state for polar molecular fluids: II. Fluid mixtures. Geochimica et Cosmochimica Acta 67, 2415-2425.
show that pressures higher than 2 GPa would cause immiscibility in the salt-free H2O-CH4 system at 450 °C. In addition to pressure, the addition of salt such as NaCl, KCl, MgCl2, and/or CaCl2 can also effectively expand the C-H-O fluid miscibility gap (Bowers and Helgeson, 1983Bowers, T.S., Helgeson, H.C. (1983) Calculation of the thermodynamic and geochemical consequences of nonideal mixing in the system H2O-CO2-NaCl on phase-relations in geologic systems - Metamorphic equilibria at high-pressures and temperatures. American Mineralogist 68, 1059-1075.
; Johnson, 1991Johnson, E.L. (1991) Experimentally determined limits for H2O-CO2-NaCl immiscibility in granulites. Geology 19, 925-928.
; Shmulovich et al., 2004Shmulovich, K.I., Graham, C.M. (2004) An experimental study of phase equilibria in the systems H2O-CO2-CaCl2 and H2O-CO2-NaCl at high pressures and temperatures (500-800 °C, 0.5-0.9 GPa): geological and geophysical applications. Contributions to Mineralogy and Petrology 146, 450-462.
; Heinrich, 2007Heinrich, W. (2007) Fluid immiscibility in metamorphic rocks. Reviews in Mineralogy and Geochemistry 65, 389-430.
). Subduction zone fluids may contain 3 to 50 wt. % NaCl equivalent based on analyses of aqueous fluid inclusions in high-pressure metamorphic rocks from subduction zones (Scambelluri and Philippot, 2001Scambelluri, M., Philippot, P. (2001) Deep fluids in subduction zones. Lithos 55, 213-227.
; Frezzotti and Ferrando, 2015Frezzotti, M.L., Ferrando, S. (2015) The chemical behavior of fluids released during deep subduction based on fluid inclusions. American Mineralogist 100, 352-377.
), or even more based on analyses of fluid inclusions in diamonds (Weiss et al., 2015Weiss, Y., McNeill, J., Pearson, D.G., Nowell, G.M., Ottley, C.J. (2015) Highly saline fluids from a subducting slab as the source for fluid-rich diamonds. Nature 524, 339-342.
). In this study, only 3 wt. % NaCl was added to the starting solution, which is at the lower end of subduction zone fluid salinity. Therefore, in conjunction with the combined effects of pressure and salt on expanding the C-H-O fluid miscibility gap, the C-H-O fluid immiscibility observed here suggests that C-H-O fluid immiscibility may also occur in subduction zones. This may particularly hold true when the slab C-H-O fluids are saturated with graphite. This is because the experiments here at 1.5 to 2.5 GPa, 600 to 700 °C, and the Co-CoO to the Re-ReO2 buffer are all saturated with graphite (Table 1), and the bulk composition and species of C-H-O fluids are fixed at graphite saturation at the given P-T-fO2 conditions (e.g., Zhang and Duan, 2009Zhang, C., Duan, Z. (2009) A model for C–O–H fluid in the Earth’s mantle. Geochimica et Cosmochimica Acta 73, 2089-2102.
). In the subducting slab, graphite could be formed by graphitisation of organic matter or by reduction of carbonates (Galvez et al., 2013Galvez, M.E., Beyssac, O., Martinez, I., Benzerara, K., Chaduteau, C., Malvoisin, B., Malavieille, J. (2013) Graphite formation by carbonate reduction during subduction. Nature Geoscience 6, 473-477.
; Stagno et al., 2015Stagno, V., Frost, D.J., McCammon, C.A., Mohseni, H., Fei, Y. (2015) The oxygen fugacity at which graphite or diamond forms from carbonate-bearing melts in eclogitic rocks. Contributions to Mineralogy and Petrology 169, 1-18.
).The occurrence of C-H-O fluid immiscibility in subduction zones is supported by the studies of fluids and fluid inclusions in high-pressure metamorphic rocks from subduction zones (Heinrich, 2007
Heinrich, W. (2007) Fluid immiscibility in metamorphic rocks. Reviews in Mineralogy and Geochemistry 65, 389-430.
; Frezzotti and Ferrando, 2015Frezzotti, M.L., Ferrando, S. (2015) The chemical behavior of fluids released during deep subduction based on fluid inclusions. American Mineralogist 100, 352-377.
). For example, Andersen et al. (1993)Andersen, T., Austrheim, H., Burke, E.A.J., Elvevold, S. (1993) Fluid-rock Interaction in the Deeper Continental Lithosphere N2 and CO2 in deep crustal fluids: evidence from the Caledonides of Norway. Chemical Geology 108, 113-132.
reported coexistence of CO2-N2 fluid inclusions and H2O-rich fluid inclusions with about 30 wt. % NaCl equivalent in eclogites of the Norwegian Caledonides, which represent immiscible fluids at peak metamorphic conditions. Fu et al. (2003aFu, B., Touret, J.L.R., Zheng, Y.F. (2003a) Remnants of premetamorphic fluid and oxygen isotopic signatures in eclogites and garnet clinopyroxenite from the Dabie-Sulu terranes, eastern China. Journal of Metamorphic Geology 21, 561-578.
,bFu, B., Touret, J.L.R., Zheng, Y.F., Jahn, B.M. (2003b) Fluid inclusions in granulites, granulitized eclogites and garnet clinopyroxenites from the Dabie-Sulu terranes, Eastern China. Lithos 70, 293-319.
) found N2-, CO2-, and CH4-rich fluid inclusions coexisting with H2O-rich fluid inclusions with 0-30 wt. % NaCl equivalent in a variety of eclogites from the Dabie-Sulu terranes of China. Based on the fluid activity calculated via mineral equilibrium between millimetre- to centimetre-scale layers in banded mafic elcogites from the Tauern Window of Austria, Selverstone et al. (1992)Selverstone, J., Franz, G., Thomas, S., Getty, S. (1992) Fluid variability in 2 GPa eclogites as an indicator of fluid behavior during subduction. Contributions to Mineralogy and Petrology 112, 341-357.
proposed that the combined effects of pressure and salt on expanding the fluid miscibility gap make it likely that blueschist and eclogite facies metamorphism in subduction zones occurs predominantly at H2O-CO2 immiscibility.It may be worth noting that N2 is a common component in the C-H-O fluids of high-pressure metamorphism, and the addition of non-polar N2 usually enlarges the C-H-O fluid immiscibility field (Heinrich, 2007
Heinrich, W. (2007) Fluid immiscibility in metamorphic rocks. Reviews in Mineralogy and Geochemistry 65, 389-430.
). Recent theoretical calculations also show that depending on the P-T-fO2 and pH buffered by minerals, carbon could be dissolved in the C-H-O fluids as organic and/or inorganic ionic species (Sverjensky et al., 2014Sverjensky, D.A., Stagno, V., Huang, F. (2014) Important role for organic carbon in subduction-zone fluids in the deep carbon cycle. Nature Geoscience 7, 909-913.
; Galvez et al., 2015Galvez, M.E., Manning, C.E., Connolly, J.A.D., Rumble, D. (2015) The solubility of rocks in metamorphic fluids: A model for rock-dominated conditions to upper mantle pressure and temperature. Earth and Planetary Science Letters 430, 486-498.
). In addition, solutes such as Al2O3 and MgO which are commonly dissolved in high-pressure metamorphic fluids are lacking in the present C-H-O fluids. How these ionic carbon species and/or solutes would affect the phase relations of C-H-O fluids at subduction zone conditions remains to be investigated in the future. Nevertheless, combined with the studies on fluids and fluid inclusions in natural high-pressure metamorphic rocks, the experimental work here demonstrates that C-H-O fluid immiscibility may indeed occur in subduction zones.top
Implications
The occurrence of immiscible C-H-O fluids may provide new insights into our understanding of decarbonation and carbon mobility in subduction zones. Firstly, C-H-O fluid immiscibility may cause extensive decarbonation in subduction zones. Previous studies show that some low-grade metamorphic reactions may cause extensive decarbonation if these reactions take place at H2O-CO2 immiscibility (Yardley and Bottrell, 1988
Yardley B.W.D., Bottrell, S.H. (1988) Immiscible fluids in metamorphism: implications of two-phase fluid flow for reaction history. Geology 16,199-202.
; Heinrich, 2007Heinrich, W. (2007) Fluid immiscibility in metamorphic rocks. Reviews in Mineralogy and Geochemistry 65, 389-430.
). Similarly, for a given decarbonation reaction in subduction zones, if it takes places at H2O-CO2 immiscibility, significant amounts of carbonates may also be consumed. Taking the decarbonation reaction of carbonate (calcite) dissolution in H2O-rich fluids (Caciagli and Manning, 2003Caciagli, N.C., Manning, C.E. (2003) The solubility of calcite in water at 6–16 kbar and 500–800 °C. Contributions to Mineralogy and Petrology 146, 275-285.
) as an exampleEq. 3
CaCO3 (calcite) + H2O = Ca2+ + 2OH- + CO2
and assuming that this reaction takes place at H2O-CO2 immiscibility in subduction zones, extensive slab decarbonation must occur if the H2O-rich fluids can be segregated from the CO2-rich fluids and react further with carbonates. Available studies show that at high pressures, the CO2-rich fluids have higher dihedral angles and density than the H2O-rich fluids, indicating that the H2O-rich fluids can be effectively segregated from the CO2-rich fluids (see Supplementary Information). Furthermore, the ascending H2O-rich fluids in the subducting slab would experience decompression and heating, and the CO2 activity in the H2O-rich fluids usually decreases with increasing temperature or decreasing pressure (Kerrick and Jacobs, 1981
Kerrick, D.M., Jacobs, G.K. (1981) A modified Redlich-Kwong equation for H2O, CO2 and H2O-CO2 mixtures at elevated pressures and temperatures. American Journal of Science 281, 735–767.
; Aranovich and Newton, 1999Aranovich, L.Y., Newton, R.C. (1999) Experimental determination of CO2-H2O activity-composition relations at 600-1000 °C and 6-14 kbar by reversed decarbonation and dehydration reactions. American Mineralogist 84, 1319-1332.
). Therefore, the segregating H2O-rich fluids could further react with carbonates during ascent, causing more decarbonation and the formation of immiscible CO2-rich fluids.Secondly, immiscible CO2-rich fluids may be an important agent for the transfer of slab carbon to the mantle wedge. The formation of immiscible CO2-rich fluids due to slab decarbonation and C-H-O fluid immiscibility indicates that a significant amount of slab carbon may be transferred to the mantle wedge in the form of CO2-rich fluids. In subduction zones, the CO2-rich fluids could be mobilised to the mantle wedge along cracks, veins, shear zones, or fold hinges (see Supplementary Information). As a result, the actual mass of slab carbon transferred to the mantle wedge should be considerably larger than the mass of carbon apparently dissolved in the H2O-rich fluids. Therefore, C-H-O fluid immiscibility in subduction zones may provide an important but hitherto unappreciated mechanism for the transfer of slab carbon to the mantle wedge.
top
Acknowledgements
Stefan Übelhack and Andreas Audétat are thanked for machining the perfect sample capsules and providing the HF-etched quartz crystals. Xu Chu and I-Ming Chou are thanked for helpful discussion and James Eguchi is thanked for both discussion and the correction of English. Constructive reviews by Katy Evans, Fabrizio Nestola, Kate Kiseeva, four anonymous reviewers, and the journal editor Helen Williams greatly improved this paper. The support from the Strategic Priority Research Program (B) of the Chinese Academy of Sciences (XDB18020301) and the Elite Network Bavaria (ENB) program is greatly appreciated.
Editor: Helen Williams
top
References
Abstract | Introduction | Results | Discussion | Implications | Acknowledgements | References | Supplementary InformationAgue, J., Nicolescu, S. (2014) Carbon dioxide released from subduction zones by fluid-mediated reactions. Nature Geoscience 7, 355-360.

Among these are metamorphic decarbonation (Poli et al., 2009), carbonate dissolution in the dehydrating fluids as gas molecules and/or ionic carbon species (Caciagli and Manning, 2003; Frezzotti et al., 2011; Sverjensky et al., 2014), infiltrating fluids induced decarbonation (Kerrick and Connolly, 2001; Gorman et al., 2006; Bebout and Penniston-Dorland, 2016), and decarbonation accompanied by silicate precipitation via fluid-carbonate reaction (Ague and Nicolescu, 2014).
View in article
For example, Galvez et al. (2013) proposed that the reaction between serpentinite-derived fluids and carbonates may cause the formation of graphite and provide an important mechanism for the deep subduction of carbon; whereas Ague and Nicolescu (2014) showed that fluid-carbonate reaction may cause significant decarbonation and efficient transfer of slab carbon to the mantle wedge.
View in article
Andersen, T., Austrheim, H., Burke, E.A.J., Elvevold, S. (1993) Fluid-rock Interaction in the Deeper Continental Lithosphere N2 and CO2 in deep crustal fluids: evidence from the Caledonides of Norway. Chemical Geology 108, 113-132.

For example, Andersen et al. (1993) reported coexistence of CO2-N2 fluid inclusions and H2O-rich fluid inclusions with about 30 wt. % NaCl equivalent in eclogites of the Norwegian Caledonides, which represent immiscible fluids at peak metamorphic conditions.
View in article
Aranovich, L.Y., Newton, R.C. (1999) Experimental determination of CO2-H2O activity-composition relations at 600-1000 °C and 6-14 kbar by reversed decarbonation and dehydration reactions. American Mineralogist 84, 1319-1332.

Furthermore, the ascending H2O-rich fluids in the subducting slab would experience decompression and heating, and the CO2 activity in the H2O-rich fluids usually decreases with increasing temperature or decreasing pressure (Kerrick and Jacobs, 1981; Aranovich and Newton, 1999).
View in article
Bali, E., Audétat, A., Keppler, H. (2013) Water and hydrogen are immiscible in Earth's mantle. Nature 495, 220-222.

Two previous studies showed that pressure can also expand the miscibility gap of the H2O-H2 and H2O-CH4 systems (Churakov and Gottschalk, 2003; Bali et al., 2013), but presently no experiments have been performed on the C-H-O-salt system at mantle conditions.
View in article
This is in good agreement with one recent experimental study (Bali et al., 2013), which shows that the critical temperature of the H2O-H2 system increases from below 400 °C at 0.25 GPa to 1050 °C at 2.6 GPa.
View in article
Bebout, G.E., Penniston-Dorland, S.C. (2016) Fluid and mass transfer at subduction interfaces—The field metamorphic record. Lithos 240, 228-258.

Among these are metamorphic decarbonation (Poli et al., 2009), carbonate dissolution in the dehydrating fluids as gas molecules and/or ionic carbon species (Caciagli and Manning, 2003; Frezzotti et al., 2011; Sverjensky et al., 2014), infiltrating fluids induced decarbonation (Kerrick and Connolly, 2001; Gorman et al., 2006; Bebout and Penniston-Dorland, 2016), and decarbonation accompanied by silicate precipitation via fluid-carbonate reaction (Ague and Nicolescu, 2014).
View in article
Berkesi, M., Hidas, K., Guzmics, T., Dubessy, J., Bodnar, R., Szabo, C., Vajna, B., Tsunogae, T. (2009) Detection of small amounts of H2O in CO2-rich fluid inclusions using Raman spectroscopy. Journal of Raman Spectroscopy 40, 1461-1463.

The corresponding Type-2 fluid inclusions are pure gases of H2+CH4+C2H6, CH4+CO2, or CO2 without detectable water at room temperatures (Fig. 2b), although a thin film of water may exist on the inner surface of the fluid inclusions (Berkesi et al., 2009; Frezzotti et al., 2012).
View in article
Bowers, T.S., Helgeson, H.C. (1983) Calculation of the thermodynamic and geochemical consequences of nonideal mixing in the system H2O-CO2-NaCl on phase-relations in geologic systems - Metamorphic equilibria at high-pressures and temperatures. American Mineralogist 68, 1059-1075.

The addition of salt, such as NaCl or CaCl2, may yield an extensive region of immiscibility in the H2O-CH4/CO2-salt system at crustal conditions (Bowers and Helgeson, 1983; Heinrich, 2007; Manning et al., 2013).
View in article
In addition to pressure, the addition of salt such as NaCl, KCl, MgCl2, and/or CaCl2 can also effectively expand the C-H-O fluid miscibility gap (Bowers and Helgeson, 1983; Johnson, 1991; Shmulovich et al., 2004; Heinrich, 2007).
View in article
Caciagli, N.C., Manning, C.E. (2003) The solubility of calcite in water at 6–16 kbar and 500–800 °C. Contributions to Mineralogy and Petrology 146, 275-285.

Among these are metamorphic decarbonation (Poli et al., 2009), carbonate dissolution in the dehydrating fluids as gas molecules and/or ionic carbon species (Caciagli and Manning, 2003; Frezzotti et al., 2011; Sverjensky et al., 2014), infiltrating fluids induced decarbonation (Kerrick and Connolly, 2001; Gorman et al., 2006; Bebout and Penniston-Dorland, 2016), and decarbonation accompanied by silicate precipitation via fluid-carbonate reaction (Ague and Nicolescu, 2014).
View in article
Taking the decarbonation reaction of carbonate (calcite) dissolution in H2O-rich fluids (Caciagli and Manning, 2003) as an example CaCO3 (calcite) + H2O = Ca2+ + 2OH- + CO2 and assuming that this reaction takes place at H2O-CO2 immiscibility in subduction zones, extensive slab decarbonation must occur if the H2O-rich fluids can be segregated from the CO2-rich fluids and react further with carbonates.
View in article
Churakov, S., Gottschalk, M. (2003) Perturbation theory based equation of state for polar molecular fluids: II. Fluid mixtures. Geochimica et Cosmochimica Acta 67, 2415-2425.

However, despite significant progress in experiments and thermodynamic modelling on C-H-O fluids (e.g., Churakov and Gottschalk, 2003; Heinrich, 2007; Zhang and Duan, 2009; Manning et al., 2013), our understanding of the nature of mantle C-H-O fluids is heavily hampered by the lack of experiments at relevant conditions.
View in article
Two previous studies showed that pressure can also expand the miscibility gap of the H2O-H2 and H2O-CH4 systems (Churakov and Gottschalk, 2003; Bali et al., 2013), but presently no experiments have been performed on the C-H-O-salt system at mantle conditions.
View in article
Theoretical calculations also indicate the expansion of the fluid miscibility gap with pressure; Churakov and Gottschalk (2003) show that pressures higher than 2 GPa would cause immiscibility in the salt-free H2O-CH4 system at 450 °C.
View in article
Dasgupta, R., Hirschmann, M.M. (2010) The deep carbon cycle and melting in Earth's interior. Earth and Planetary Science Letters 298, 1-13.

Thermodynamic simulations and experimental studies demonstrate that carbonate minerals are stable along most subduction P-T paths and up to 90 % of the carbon in subduction zones should be subducted into the deep mantle (Kerrick and Connolly, 2001; Dasgupta and Hirschmann, 2010).
View in article
However, there is compelling evidence suggesting that about 20−80 % of carbon is removed from the downgoing slab and returned to Earth’s surface by arc volcanism (Dasgupta and Hirschmann, 2010; Johnston et al., 2011; Kelemen and Manning, 2015).
View in article
Frezzotti, M.L., Ferrando, S. (2015) The chemical behavior of fluids released during deep subduction based on fluid inclusions. American Mineralogist 100, 352-377.

Subduction zone fluids may contain 3 to 50 wt. % NaCl equivalent based on analyses of aqueous fluid inclusions in high-pressure metamorphic rocks from subduction zones (Scambelluri and Philippot, 2001; Frezzotti and Ferrando, 2015), or even more based on analyses of fluid inclusions in diamonds (Weiss et al., 2015).
View in article
The occurrence of C-H-O fluid immiscibility in subduction zones is supported by the studies of fluids and fluid inclusions in high-pressure metamorphic rocks from subduction zones (Heinrich, 2007; Frezzotti and Ferrando, 2015).
View in article
Frezzotti, M., Selverstone, J., Sharp, Z., Compagnoni, R. (2011) Carbonate dissolution during subduction revealed by diamond-bearing rocks from the Alps. Nature Geoscience 4, 703-706.

Among these are metamorphic decarbonation (Poli et al., 2009), carbonate dissolution in the dehydrating fluids as gas molecules and/or ionic carbon species (Caciagli and Manning, 2003; Frezzotti et al., 2011; Sverjensky et al., 2014), infiltrating fluids induced decarbonation (Kerrick and Connolly, 2001; Gorman et al., 2006; Bebout and Penniston-Dorland, 2016), and decarbonation accompanied by silicate precipitation via fluid-carbonate reaction (Ague and Nicolescu, 2014).
View in article
Frezzotti, M.L., Ferrando, S., Tecce, F., Castelli, D. (2012) Water content and nature of solutes in shallow-mantle fluids from fluid inclusions. Earth and Planetary Science Letters 351-352, 70-83.

The corresponding Type-2 fluid inclusions are pure gases of H2+CH4+C2H6, CH4+CO2, or CO2 without detectable water at room temperatures (Fig. 2b), although a thin film of water may exist on the inner surface of the fluid inclusions (Berkesi et al., 2009; Frezzotti et al., 2012).
View in article
Fu, B., Touret, J.L.R., Zheng, Y.F. (2003a) Remnants of premetamorphic fluid and oxygen isotopic signatures in eclogites and garnet clinopyroxenite from the Dabie-Sulu terranes, eastern China. Journal of Metamorphic Geology 21, 561-578.

Fu et al. (2003a,b) found N2-, CO2-, and CH4-rich fluid inclusions coexisting with H2O-rich fluid inclusions with 0-30 wt. % NaCl equivalent in a variety of eclogites from the Dabie-Sulu terranes of China.
View in article
Fu, B., Touret, J.L.R., Zheng, Y.F., Jahn, B.M. (2003b) Fluid inclusions in granulites, granulitized eclogites and garnet clinopyroxenites from the Dabie-Sulu terranes, Eastern China. Lithos 70, 293-319.

Fu et al. (2003a,b) found N2-, CO2-, and CH4-rich fluid inclusions coexisting with H2O-rich fluid inclusions with 0-30 wt. % NaCl equivalent in a variety of eclogites from the Dabie-Sulu terranes of China.
View in article
Galvez, M.E., Beyssac, O., Martinez, I., Benzerara, K., Chaduteau, C., Malvoisin, B., Malavieille, J. (2013) Graphite formation by carbonate reduction during subduction. Nature Geoscience 6, 473-477.

For example, Galvez et al. (2013) proposed that the reaction between serpentinite-derived fluids and carbonates may cause the formation of graphite and provide an important mechanism for the deep subduction of carbon; whereas Ague and Nicolescu (2014) showed that fluid-carbonate reaction may cause significant decarbonation and efficient transfer of slab carbon to the mantle wedge.
View in article
In the subducting slab, graphite could be formed by graphitisation of organic matter or by reduction of carbonates (Galvez et al., 2013; Stagno et al., 2015).
View in article
Galvez, M.E., Manning, C.E., Connolly, J.A.D., Rumble, D. (2015) The solubility of rocks in metamorphic fluids: A model for rock-dominated conditions to upper mantle pressure and temperature. Earth and Planetary Science Letters 430, 486-498.

Recent theoretical calculations also show that depending on the P-T-fO2 and pH buffered by minerals, carbon could be dissolved in the C-H-O fluids as organic and/or inorganic ionic species (Sverjensky et al., 2014; Galvez et al., 2015).
View in article
Gorman, P.J., Kerrick, D.M., Connolly, J.A.D. (2006) Modeling open system metamorphic decarbonation of subducting slabs. Geochemistry, Geophysics, Geosystems 7, doi: 10.1029/2005gc001125.

Among these are metamorphic decarbonation (Poli et al., 2009), carbonate dissolution in the dehydrating fluids as gas molecules and/or ionic carbon species (Caciagli and Manning, 2003; Frezzotti et al., 2011; Sverjensky et al., 2014), infiltrating fluids induced decarbonation (Kerrick and Connolly, 2001; Gorman et al., 2006; Bebout and Penniston-Dorland, 2016), and decarbonation accompanied by silicate precipitation via fluid-carbonate reaction (Ague and Nicolescu, 2014).
View in article
Heinrich, W. (2007) Fluid immiscibility in metamorphic rocks. Reviews in Mineralogy and Geochemistry 65, 389-430.

However, despite significant progress in experiments and thermodynamic modelling on C-H-O fluids (e.g., Churakov and Gottschalk, 2003; Heinrich, 2007; Zhang and Duan, 2009; Manning et al., 2013), our understanding of the nature of mantle C-H-O fluids is heavily hampered by the lack of experiments at relevant conditions.
View in article
Previous studies assumed that H2O and gases of CH4, CO2, or H2 are fully miscible at conditions of most of the crust and the entire mantle (e.g., Heinrich, 2007; Manning et al., 2013).
View in article
The addition of salt, such as NaCl or CaCl2, may yield an extensive region of immiscibility in the H2O-CH4/CO2-salt system at crustal conditions (Bowers and Helgeson, 1983; Heinrich, 2007; Manning et al., 2013).
View in article
In addition to pressure, the addition of salt such as NaCl, KCl, MgCl2, and/or CaCl2 can also effectively expand the C-H-O fluid miscibility gap (Bowers and Helgeson, 1983; Johnson, 1991; Shmulovich et al., 2004; Heinrich, 2007).
View in article
The occurrence of C-H-O fluid immiscibility in subduction zones is supported by the studies of fluids and fluid inclusions in high-pressure metamorphic rocks from subduction zones (Heinrich, 2007; Frezzotti and Ferrando, 2015).
View in article
It may be worth noting that N2 is a common component in the C-H-O fluids of high-pressure metamorphism, and the addition of non-polar N2 usually enlarges the C-H-O fluid immiscibility field (Heinrich, 2007).
View in article
Previous studies show that some low-grade metamorphic reactions may cause extensive decarbonation if these reactions take place at H2O-CO2 immiscibility (Yardley and Bottrell, 1988; Heinrich, 2007).
View in article
Johnson, E.L. (1991) Experimentally determined limits for H2O-CO2-NaCl immiscibility in granulites. Geology 19, 925-928.

However, at deep crustal conditions the salt concentration may need to be more than 20 wt. % to yield fluid immiscibility if CO2 or CH4 mole fraction in the system is less than 0.5 (Johnson, 1991; Shmulovich et al., 2004).
View in article
In addition to pressure, the addition of salt such as NaCl, KCl, MgCl2, and/or CaCl2 can also effectively expand the C-H-O fluid miscibility gap (Bowers and Helgeson, 1983; Johnson, 1991; Shmulovich et al., 2004; Heinrich, 2007).
View in article
Johnston, F.K., Turchyn, A.V., Edmonds, M. (2011) Decarbonation efficiency in subduction zones: Implications for warm Cretaceous climates. Earth and Planetary Science Letters 303, 143-152.

However, there is compelling evidence suggesting that about 20−80 % of carbon is removed from the downgoing slab and returned to Earth’s surface by arc volcanism (Dasgupta and Hirschmann, 2010; Johnston et al., 2011; Kelemen and Manning, 2015).
View in article
Kelemen, P.B., Manning, C.E. (2015) Reevaluating carbon fluxes in subduction zones, what goes down, mostly comes up. Proceedings of the National Academy of Science USA 112, E3997-E4006.

However, there is compelling evidence suggesting that about 20−80 % of carbon is removed from the downgoing slab and returned to Earth’s surface by arc volcanism (Dasgupta and Hirschmann, 2010; Johnston et al., 2011; Kelemen and Manning, 2015).
View in article
Kerrick, D.M., Jacobs, G.K. (1981) A modified Redlich-Kwong equation for H2O, CO2 and H2O-CO2 mixtures at elevated pressures and temperatures. American Journal of Science 281, 735–767.

Furthermore, the ascending H2O-rich fluids in the subducting slab would experience decompression and heating, and the CO2 activity in the H2O-rich fluids usually decreases with increasing temperature or decreasing pressure (Kerrick and Jacobs, 1981; Aranovich and Newton, 1999).
View in article
Kerrick, D., Connolly, J. (2001) Metamorphic devolatilization of subducted marine sediments and the transport of volatiles into the Earth's mantle. Nature 411, 293-296.

Thermodynamic simulations and experimental studies demonstrate that carbonate minerals are stable along most subduction P-T paths and up to 90 % of the carbon in subduction zones should be subducted into the deep mantle (Kerrick and Connolly, 2001; Dasgupta and Hirschmann, 2010).
View in article
Among these are metamorphic decarbonation (Poli et al., 2009), carbonate dissolution in the dehydrating fluids as gas molecules and/or ionic carbon species (Caciagli and Manning, 2003; Frezzotti et al., 2011; Sverjensky et al., 2014), infiltrating fluids induced decarbonation (Kerrick and Connolly, 2001; Gorman et al., 2006; Bebout and Penniston-Dorland, 2016), and decarbonation accompanied by silicate precipitation via fluid-carbonate reaction (Ague and Nicolescu, 2014).
View in article
Manning, C.E., Shock, E.L., Sverjensky, D.A. (2013) The Chemistry of Carbon in Aqueous Fluids at Crustal and Upper-Mantle Conditions: Experimental and Theoretical Constraints. Reviews in Mineralogy and Geochemistry 75, 109-148.

However, despite significant progress in experiments and thermodynamic modelling on C-H-O fluids (e.g., Churakov and Gottschalk, 2003; Heinrich, 2007; Zhang and Duan, 2009; Manning et al., 2013), our understanding of the nature of mantle C-H-O fluids is heavily hampered by the lack of experiments at relevant conditions.
View in article
Previous studies assumed that H2O and gases of CH4, CO2, or H2 are fully miscible at conditions of most of the crust and the entire mantle (e.g., Heinrich, 2007; Manning et al., 2013).
View in article
The addition of salt, such as NaCl or CaCl2, may yield an extensive region of immiscibility in the H2O-CH4/CO2-salt system at crustal conditions (Bowers and Helgeson, 1983; Heinrich, 2007; Manning et al., 2013).
View in article
Poli, S., Franzolin, E., Fumagalli, P., Crottini, A. (2009) The transport of carbon and hydrogen in subducted oceanic crust: An experimental study to 5 GPa. Earth and Planetary Science Letters 278, 350-360.

Among these are metamorphic decarbonation (Poli et al., 2009), carbonate dissolution in the dehydrating fluids as gas molecules and/or ionic carbon species (Caciagli and Manning, 2003; Frezzotti et al., 2011; Sverjensky et al., 2014), infiltrating fluids induced decarbonation (Kerrick and Connolly, 2001; Gorman et al., 2006; Bebout and Penniston-Dorland, 2016), and decarbonation accompanied by silicate precipitation via fluid-carbonate reaction (Ague and Nicolescu, 2014).
View in article
Scambelluri, M., Philippot, P. (2001) Deep fluids in subduction zones. Lithos 55, 213-227.

Subduction zone fluids may contain 3 to 50 wt. % NaCl equivalent based on analyses of aqueous fluid inclusions in high-pressure metamorphic rocks from subduction zones (Scambelluri and Philippot, 2001; Frezzotti and Ferrando, 2015), or even more based on analyses of fluid inclusions in diamonds (Weiss et al., 2015).
View in article
Selverstone, J., Franz, G., Thomas, S., Getty, S. (1992) Fluid variability in 2 GPa eclogites as an indicator of fluid behavior during subduction. Contributions to Mineralogy and Petrology 112, 341-357.

Based on the fluid activity calculated via mineral equilibrium between millimetre- to centimetre-scale layers in banded mafic elcogites from the Tauern Window of Austria, Selverstone et al. (1992) proposed that the combined effects of pressure and salt on expanding the fluid miscibility gap make it likely that blueschist and eclogite facies metamorphism in subduction zones occurs predominantly at H2O-CO2 immiscibility.
View in article
Shmulovich, K.I., Graham, C.M. (2004) An experimental study of phase equilibria in the systems H2O-CO2-CaCl2 and H2O-CO2-NaCl at high pressures and temperatures (500-800 °C, 0.5-0.9 GPa): geological and geophysical applications. Contributions to Mineralogy and Petrology 146, 450-462.

However, at deep crustal conditions the salt concentration may need to be more than 20 wt. % to yield fluid immiscibility if CO2 or CH4 mole fraction in the system is less than 0.5 (Johnson, 1991; Shmulovich et al., 2004).
View in article
In addition to pressure, the addition of salt such as NaCl, KCl, MgCl2, and/or CaCl2 can also effectively expand the C-H-O fluid miscibility gap (Bowers and Helgeson, 1983; Johnson, 1991; Shmulovich et al., 2004; Heinrich, 2007).
View in article
Stagno, V., Frost, D.J., McCammon, C.A., Mohseni, H., Fei, Y. (2015) The oxygen fugacity at which graphite or diamond forms from carbonate-bearing melts in eclogitic rocks. Contributions to Mineralogy and Petrology 169, 1-18.

In the subducting slab, graphite could be formed by graphitisation of organic matter or by reduction of carbonates (Galvez et al., 2013; Stagno et al., 2015).
View in article
Sverjensky, D.A., Stagno, V., Huang, F. (2014) Important role for organic carbon in subduction-zone fluids in the deep carbon cycle. Nature Geoscience 7, 909-913.

Among these are metamorphic decarbonation (Poli et al., 2009), carbonate dissolution in the dehydrating fluids as gas molecules and/or ionic carbon species (Caciagli and Manning, 2003; Frezzotti et al., 2011; Sverjensky et al., 2014), infiltrating fluids induced decarbonation (Kerrick and Connolly, 2001; Gorman et al., 2006; Bebout and Penniston-Dorland, 2016), and decarbonation accompanied by silicate precipitation via fluid-carbonate reaction (Ague and Nicolescu, 2014).
View in article
Recent theoretical calculations also show that depending on the P-T-fO2 and pH buffered by minerals, carbon could be dissolved in the C-H-O fluids as organic and/or inorganic ionic species (Sverjensky et al., 2014; Galvez et al., 2015).
View in article
Weiss, Y., McNeill, J., Pearson, D.G., Nowell, G.M., Ottley, C.J. (2015) Highly saline fluids from a subducting slab as the source for fluid-rich diamonds. Nature 524, 339-342.

Subduction zone fluids may contain 3 to 50 wt. % NaCl equivalent based on analyses of aqueous fluid inclusions in high-pressure metamorphic rocks from subduction zones (Scambelluri and Philippot, 2001; Frezzotti and Ferrando, 2015), or even more based on analyses of fluid inclusions in diamonds (Weiss et al., 2015).
View in article
Yardley B.W.D., Bottrell, S.H. (1988) Immiscible fluids in metamorphism: implications of two-phase fluid flow for reaction history. Geology 16,199-202.

Previous studies show that some low-grade metamorphic reactions may cause extensive decarbonation if these reactions take place at H2O-CO2 immiscibility (Yardley and Bottrell, 1988; Heinrich, 2007).
View in article
Zhang, C., Duan, Z. (2009) A model for C–O–H fluid in the Earth’s mantle. Geochimica et Cosmochimica Acta 73, 2089-2102.

However, despite significant progress in experiments and thermodynamic modelling on C-H-O fluids (e.g., Churakov and Gottschalk, 2003; Heinrich, 2007; Zhang and Duan, 2009; Manning et al., 2013), our understanding of the nature of mantle C-H-O fluids is heavily hampered by the lack of experiments at relevant conditions.
View in article
This is because the experiments here at 1.5 to 2.5 GPa, 600 to 700 °C, and the Co-CoO to the Re-ReO2 buffer are all saturated with graphite (Table 1), and the bulk composition and species of C-H-O fluids are fixed at graphite saturation at the given P-T-fO2 conditions (e.g., Zhang and Duan, 2009).
View in article
top
Supplementary Information
Methods
The starting solution includes 78.5 wt. % H2O, 18.5 wt. % formic acid (HCO2H), and 3 wt. % NaCl. The aim is to obtain H2O-rich C-H-O fluids with a low salt concentration. As observed in some high-pressure metamorphic rocks from subduction zones, the salt concentration in subduction zone fluids could be between 3 and 50 wt. % (Scambelluri and Philippot, 2001; Frezzotti and Ferrando, 2015). At high P-T conditions, formic acid first decomposes into H2O, CO, CO2, and H2 (Fujii et al., 2012), but the final C-H-O fluid speciation present during the run primarily depends on the fO2 imposed. The experiments at 0.2 GPa and 700 °C were carried out in rapid quench cold-seal pressure vessels housed at Bayerisches Geoinstitut, University Bayreuth, Germany, with water as the pressure media. Overall temperature and pressure uncertainties in the recorded pressure and temperature are less than 50 bar and 15 °C. The starting solution was loaded together with a piece of quartz crystal into an Au or Pt capsule (Fig. S-1a,b). The pressure vessels allow for in situ cracking on the quartz crystal, so that the timing of fluid inclusion formation can be controlled, as well as to ensure that the entrapped fluids are in equilibrium with the oxygen buffer (Li and Audétat, 2009; Li et al., 2009; Li and Keppler, 2014). Oxygen fugacity (fO2) at the fayalite-magnetite-quartz (FMQ) buffer was controlled by loading a mixture of synthetic fayalite, magnetite, and SiO2 powder into the sample Au capsule (Fig. S-1a), and fO2 at the Ni-NiO buffer was controlled by placing an unsealed Au capsule filled with mixed Ni and NiO powder below the sample Pt capsule (Fig. S-1b). The presence of all the buffer materials after quench was confirmed using X-Ray diffraction.
The experiments at 1.5 to 2.5 GPa and 600 to 700 °C were carried out in an end-loaded piston cylinder apparatus housed at Bayerisches Geoinstitut, University Bayreuth, Germany, with an NaCl-MgO assembly and graphite heater. Overall temperature and pressure uncertainties in the recorded pressures and temperatures are about 0.1 GPa and 10 °C. A modified double capsule technique (Li and Keppler, 2014) was used to encapsulate the starting solution and quartz crystal and to buffer the sample at different fO2 (Fig. S-1c). The quartz crystal was HF-etched in order to effectively entrap fluids as fluid inclusions (Li and Audétat, 2009). The inner sample capsule was made of Pt95Rh05 and sealed by arc welding using a method developed by Audétat and Bali (2010). The outer capsule was made of Fe, Co, or Ni metal, which can be mechanically sealed under high pressures. The space between the two capsules was filled with corresponding oxides (FeO, CoO, or NiO) and water. To achieve a relatively high fO2, the conventional double capsule technique (Chou, 1987) was used. In this technique, the inner Pt95Rh05 capsule was directly sealed in an outer Pt95Rh05 capsule and the space between was filled with Re-ReO2 powder and water (Fig. S-1d).

Figure S-1 Sketch of the four experimental capsule setups used in this study. (a, b) Panels are for the experiments performed at 0.2 GPa. (c, d) Panels are for the experiments performed at 1.5 to 2.5 GPa. The wall thickness of Au or Pt capsule in panels (a, b) and the wall thickness of the inner Pt95Rh05 capsule in panels (c, d) are about 0.15 mm and 0.2 mm, respectively. FMQ = fayalite + magnetite + quartz. See text for more details.
The use of piston cylinder apparatus at pressures of 1.5 to 2.5 GPa, rather than the cold-seal pressure vessels used at 0.2 GPa, is due to the limited pressures (≤0.25 GPa) that can be achieved by cold-seal pressure vessels. Numerous studies have demonstrated that the presence or absence of immiscible C-H-N-O-salt fluids at high pressures cannot be due to the different pressure vessels used, but mainly due to the variation of pressure, temperature, and/or salt concentration (e.g., Lamb et al., 2002; Shmulovich et al., 2004; Li et al., 2009; Bali et al., 2013; Li and Keppler, 2014).
Laser Raman was used to determine the C-H-O species in the fluid inclusions. Micro-Raman spectra of fluid inclusions were recorded in back-scattering geometry using a Horiba LabRAM HR UV spectrometer with CCD detector, 1800 mm-1 grating, 50× objective, and confocal mode, housed at Bayerisches Geoinstitut, University Bayreuth, Germany. Excitation was performed using the 514.5 nm line of an Ar+ ion laser with an output power of 0.1 W. Most spectra were collected from 200 to 4500 cm-1 with acquisition time 2 × 60 s for each range. A confocal pinhole of 100 µm was used, which limits spectral resolution to 3.5 cm-1. The use of a laser output power of 0.015 W and acquisition time 2 × 10 s yielded the same results of C-H-O species, indicating the preservation of C-H-O species during Raman analyses with a laser output power of 0.1 W.
Equilibrium and Sample fO2
Several lines of evidence suggest that equilibrium should be approached in the piston cylinder experiments. First, the experiments started with a single, homogeneous fluid, but ended with two immiscible fluids. Second, the fluid species vary as a function of fO2 imposed. From low fO2 buffered by Fe-FeO to high fO2 buffered by Re-ReO2, the C-H-O species change from H2+C2H6+CH4+H2O to CO2+H2O, which indicates that the C-H-O fluids were entrapped in the quartz crystal after the equilibrium between C-H-O fluids in the inner capsule and oxygen buffer in the outer capsule was approached. Third, analysing the earliest formed fluid inclusions in the quartz crystal from run LY05 (2.5 GPa, 600 °C, Ni-NiO, and run duration of 2 days) and the latest formed fluid inclusions in the quartz crystal from run LY08 (2.5 GPa, 600 °C, Ni-NiO, and run duration of 6 days) yielded the same results (Table 1).
Since the H2O mole fraction in the starting solution is more than 0.9, the fO2 in the inner sample capsule of the experiments should approximately equal the external oxygen buffer. However, X-Ray diffraction measurements of the buffering materials show that ReO2 in run LY19 (see Table 1) was exhausted during the run. Therefore, the fO2 in this run should be below the Re-ReO2 buffer. The coexistence of graphite and CO2-rich fluid (see Table 1 and the main text) indicates that the actual fO2 in this run may be close to the C-CO2 buffer. In the runs buffered by Fe-FeO (Table 1), the fO2 in the inner capsule should be lower than the Fe-FeO buffer due to the presence of a considerable amount of H2, CH4, and C2H6 in the H2O-rich fluids (Fig. 2). In the P-T range of 1.5 to 2.5 GPa and 600 to 700 °C, the order of oxygen buffers in terms of buffering capacity is Re-ReO2 > C-CO2 > Ni-NiO > Co-CoO > Fe-FeO.
Separation of CO2-rich and H2O-rich Fluids in Subduction Zones
Available studies show that CO2-rich and the H2O-rich fluids have very different wetting properties in calcite, quartz, and olivine dominated rocks (Watson and Brenan, 1987; Holness and Graham, 1991). The dihedral angle of the CO2-rich fluids is significantly higher than that of the H2O-rich fluids, but the dihedral angles of both fluids are greater than 60°. However, high contents of dissolved salt and/or silicates have been demonstrated to be capable of decreasing the dihedral angle of the H2O-rich fluids to less than 60° (Watson and Brenan, 1987; Holness and Graham, 1991; Wanamaker and Kohlstedt, 1991); the H2O-rich fluids in deep subduction zones may dissolve up to a few tens of wt. % of silicates and NaCl (Frezzoti and Ferrando, 2015; Weiss et al., 2015). The dihedral angle of the H2O-rich fluids may also decrease to less than 60° with increasing pressure in subduction zones (Mibe et al., 1999). Therefore, it is highly likely that in the subducting slab, the H2O-rich fluids can be effectively segregated from the CO2-rich fluids. However, a large number of recent studies (Zack and John, 2007; Ague, 2014; Manea et al., 2014; Bebout and Penniston-Dorland, 2016) demonstrate that fluids in the subducting slab may be mainly mobilised as channelised fluid flow along cracks, veins, shear zones, or fold hinges. In this case, the segregation of the H2O-rich fluids from the CO2-rich fluids could take place due to the density contrast of the fluids. The density of CO2 at subduction zone conditions, calculated using the equation of state of Sterner and Pitzer (1994), is higher than that of H2O-rich subduction zone fluids at the same P-T conditions (Hack and Thompson, 2011). For example, at 620 °C and 3 GPa, the calculated density of CO2 is about 1.7 g cm-3, while the density of H2O-rich subduction zone fluids is about 1.3 g cm-3 (Hack and Thompson, 2011). The entrapment of the H2O-rich and the CO2-rich fluids in the same quartz crystal in a ~5 mm length capsule as shown in this study essentially indicates the effective segregation of these two different fluids, which suggests that these fluids are also likely to segregate in channelised fluid flow.
Supplementary Information References
Audétat, A., Bali, E. (2010) A new technique to seal volatile-rich samples into platinum capsules. European Journal of Mineralogy 22, 23-27.
Bali, E., Audétat, A., Keppler, H. (2013) Water and hydrogen are immiscible in Earth's mantle. Nature 495, 220-222.
Bebout, G.E., Penniston-Dorland, S.C. (2016) Fluid and mass transfer at subduction interfaces—The field metamorphic record. Lithos 240, 228-258.
Chou, I.-M. (1987) Oxygen buffer and hydrogen sensor techniques at elevated pressures and temperatures. Hydrothermal experimental techniques, 61-99.
Frezzotti, M.L., Ferrando, S. (2015) The chemical behavior of fluids released during deep subduction based on fluid inclusions. American Mineralogist 100, 352-377.
Fujii, T., Hayashi, R., Kawasaki, S.-I., Suzuki, A., Oshima, Y. (2012) Effects of pressure on decomposition of formic acid in sub- and super-critical water. The Journal of Supercritical Fluids 71, 114-119.
Hack, A.C., Thompson, A.B. (2011) Density and viscosity of hydrous magmas and related fluids and their role in subduction zone processes. Journal of Petrology 52, 1333-1362.
Holness, M.B., Graham, C.M. (1991) Equilibrium dihedral angles in the system H2O− CO2− NaCl-calcite, and implications for fluid flow during metamorphism. Contributions to Mineralogy and Petrology 108, 368-383.
Johnson, E.L. (1991) Experimentally determined limits for H2O-CO2-NaCl immiscibility in granulites. Geology 19, 925-928.
Lamb, W.M., McShane, C.J., Popp, R.K. (2002) Phase relations in the CH4-H2O-NaCl system at 2 kbar, 300 to 600 °C as determined using synthetic fluid inclusions. Geochimica et Cosmochimica Acta 66, 3971-3986.
Li, Y., Audétat, A. (2009) A method to synthesize large fluid inclusions in quartz at controlled times and under unfavorable growth conditions. American Mineralogist 94, 367-371.
Li, Y., Keppler, H. (2014) Nitrogen speciation in mantle and crustal fluids. Geochimica et Cosmochimica Acta 129, 13-32.
Li, Y., Audétat, A., Lerchbaumer, L., Xiong, X. (2009) Rapid Na, Cu exchange between synthetic fluid inclusions and external aqueous solutions: evidence from LA-ICP-MS analysis. Geofluids 9, 321-329.
Manea, V.C., Leeman, W.P., Gerya, T., Manea, M., Zhu, G. (2014) Subduction of fracture zones controls mantle melting and geochemical signature above slabs. Nature communications 5, 5095.
Mibe, K., Fujii, T., Yasuda, A. (1999) Control of the location of the volcanic front in island arcs by aqueous fluid connectivity in the mantle wedge. Nature 401, 259-262.
Scambelluri, M., Philippot, P. (2001) Deep fluids in subduction zones. Lithos 55, 213-227.
Shmulovich, K.I., Graham, C.M. (2004) An experimental study of phase equilibria in the systems H2O-CO2-CaCl2 and H2O-CO2-NaCl at high pressures and temperatures (500-800 °C, 0.5-0.9 GPa): geological and geophysical applications. Contributions to Mineralogy and Petrology 146, 450-462.
Sterner, S.M., Pitzer, K.S. (1994) An equation of state for carbon dioxide valid from zero to extreme pressures. Contributions to Mineralogy and Petrology 117, 362-374.
Wanamaker, B., Kohlstedt, D. (1991) The effect of melt composition on the wetting angle between silicate melts and olivine. Physics and Chemistry of Minerals 18, 26-36.
Watson, E.B., Brenan, J.M. (1987) Fluids in the lithosphere, 1. Experimentally-determined wetting characteristics of CO2-H2O fluids and their implications for fluid transport, host-rock physical properties, and fluid inclusion formation. Earth and Planetary Science Letters 85, 497-515.
Weiss, Y., McNeill, J., Pearson, D.G., Nowell, G.M., Ottley, C.J. (2015) Highly saline fluids from a subducting slab as the source for fluid-rich diamonds. Nature 524, 339-342.
Zack, T., John, T. (2007) An evaluation of reactive fluid flow and trace element mobility in subducting slabs. Chemical Geology 239, 199-216.
Figures and Tables
Table 1 Summary of experimental conditions and results.
Run No | P | T | Duration | Oxygen buffer | Immiscible fluids | Graphite saturation | aFluid composition | |
| GPa | °C | days | | ||||
LY13 | 0.2 | 700 | 3 | FMQ | No | No | H2O-CO2 | |
LY14 | 0.2 | 700 | 3 | Ni-NiO | No | No | H2O-CO2 | |
| Type-1 | Type-2 | ||||||
LY01 | 1.5 | 600 | 3 | Fe-FeO | Yes | No | H2O-CH4-C2H6 | CH4-H2-C2H6 |
LY02 | 2.5 | 600 | 3 | Fe-FeO | Yes | No | H2O-CH4-C2H6 | CH4-H2-C2H6 |
LY05 | 2.5 | 600 | 2 | Ni-NiO | Yes | Yes | H2O-CH4-CO2 | CH4-CO2 |
LY08 | 2.5 | 600 | 6 | Ni-NiO | Yes | Yes | H2O-CH4-CO2 | CH4-CO2 |
LY04 | 2.5 | 700 | 2 | Ni-NiO | Yes | Yes | H2O-CH4-CO2 | CH4-CO2 |
LY21 | 2.5 | 700 | 2.5 | Co-CoO | Yes | Yes | H2O-CH4-CO2 | CH4-CO2 |
LY19 | 2.5 | 700 | 2 | Re-ReO2 | Yes | Yes | H2O-CO2 | CO2 |
a The fluid composition was determined by Raman spectroscopy. In each run, 3 wt. % NaCl was added to the starting solution.
Note that the oxygen fugacity in run LY19 may be close to the C-CO2 buffer. See text for more details.

Figure 1 Representative synthetic fluid inclusions in quartz formed at 0.2 to 2.5 GPa, 600 to 700 °C, and fO2 buffered by the Fe-FeO to the Re-ReO2 buffer. (a) At 0.2 GPa, only one type of fluid inclusion was observed and these fluid inclusions show a constant vapour/liquid ratio. At 1.5 and 2.5 GPa, three different types of fluid inclusions were observed: (b, c, d) Type-1 fluid inclusions are water-rich and show weak optical contrast to quartz; (b, c, d, e) Type-2 fluid inclusions are nearly pure gases and show strong optical contrast to quartz; and (c) Type-3 fluid inclusions are mixtures of Type-1 and Type-2 fluids and show variable vapour/liquid ratios. (d, f) Graphite occurs in fluid inclusions and also as solid inclusions in quartz. The coexistence of Type-1 and Type-2, together with Type-3, fluid inclusions at 1.5 and 2.5 GPa demonstrates that immiscible fluid phases must have occurred during the run. The red spots refer to the laser spots of Raman measurements, and representative Raman spectra are shown in Figure 2.

Figure 2 Representative Raman spectra of synthetic fluid inclusions in quartz formed at 0.2 to 2.5 GPa, 600 to 700 °C, and fO2 buffered by the Fe-FeO to the Re-ReO2 buffer. At 0.2 GPa, 700 °C, and fO2 at the FMQ buffer, CO2 was the only detectable carbon species in fluids. At 2.5 GPa, (a) Type-1 fluid inclusions are rich in H2O but with detectable CH4+C2H6, CH4+CO2, and CO2; (b) the corresponding Type-2 fluid inclusions are nearly pure CH4+H2+C2H6, CH4+CO2, and CO2. Note that the gas species vary as a function of fO2. Also note that the fO2 in run LY19 (ReReO2-700 °C-2.5 GPa) buffered by Re-ReO2 may be close to the C-CO2 buffer (see Supplementary Information). The Raman spectra of one graphite inclusion in quartz is also shown in panel (a). The peak positions are about 1285 cm-1 and 1388 cm-1 for CO2, 2917 cm-1 for CH4, 4150 cm-1 for H2, 2956 cm-1 for C2H6, and 3072 cm-1 for aromatics. The small peaks at about 2331 cm-1 and 1555 cm-1 could be N2 and O2 from the air, respectively. Aromatics might be produced during quench.
Supplementary Figures and Tables

Figure S-1 Sketch of the four experimental capsule setups used in this study. (a, b) Panels are for the experiments performed at 0.2 GPa. (c, d) Panels are for the experiments performed at 1.5 to 2.5 GPa. The wall thickness of Au or Pt capsule in panels (a, b) and the wall thickness of the inner Pt95Rh05 capsule in panels (c, d) are about 0.15 mm and 0.2 mm, respectively. FMQ = fayalite + magnetite + quartz. See text for more details.